Posters in the hallway
This page contains more information about the 17 posters in the hallway
Poster 1
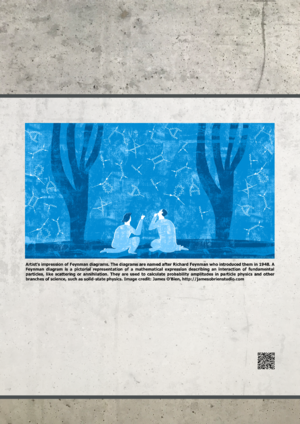
Artist's impression of Feynman diagrams
The image with the Feynman diagrams in the sky was created by the artist James O'Brien for an issue of the Quanta Magazine in 2006. We are very grateful that James gave us the permission to use the image. Check out James O'Brien's web page to see more of his beautiful illustrations and other works.
Poster 2
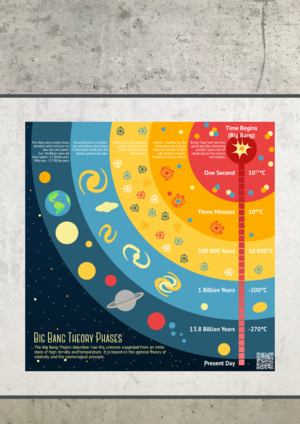
Evolution of the universe
The currently accepted Big Bang model for the evolution of the universe is called ΛCDM (cosmological constant lambda (Λ) and cold dark matter). It is based on a wealth of observational data (cosmic microwave background, abundances of elements (H, He, Li), the large-scale distribution of galaxies, accelerated expansion measured the redshift of distant galaxies), and predicts that the universe is about 13.8 billion years old. Its main components are cold dark matter, ordinary matter, and the cosmological constant that is a variant of a dark energy and drives the expansion of the universe. Particle physics contributes to a better understandig of the early, hot phase of the expanding universe and the search for an explanation for the dark matter in terms of unknown particles and fields.
Poster 3

A near-infrared image recorded by the James Webb telescope
The James Webb Space Telescope (JWST) is devoted to infrared astronomy but can also detect red and orange light from the visible spectrum. It was launched in 2021 and observes from the Lagrange Point L2 (about 1,5 million kilometers beyond the Earth's orbit) where the gravitational forces of Earth and Sun cancel each other. The instruments on the JWST cover the wavelength range between 0.6 and 28.5 microns. This makes the JWST ideal for the detection of the strongly redshifted light of distant galaxies.
Poster 4
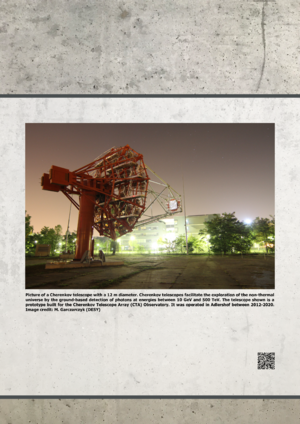
Picture of a Cherenkov Telescope with 12m diameter
The shown Cherenkov Telescope was a prototype for the Cherenkov Telescope Array (CTA) Observatory. CTA is being built on two sites in the southern (Paranal, Chile) and northern (La Palma, Spain) hemisphere, respectively. The initial installations will comprise 13 (CTA-North) and 51 (CTA-South) telesopes, respectively. CTA is the successor of Cherenkov arrays with 2-5 telescopes like HESS, MAGIC and VERITAS. These oservatories discovered more than 200 sources of very-high energy gamma rays (cf. the TeVCat catalogue) in the 20 years after 2003. It is expected that CTA will bring the source count to more than 1000. The science conducted with CTA covers galactic and extragalactic astrophysics but also fundamental physics (like indirect dark matter searches and tests for Lorentz invariance violation).
Poster 5
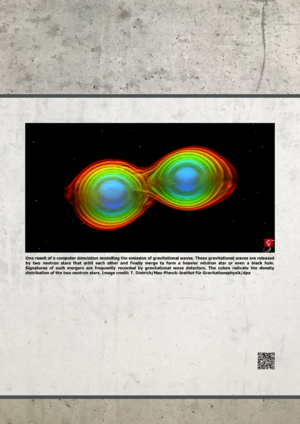
Result of a computer simulation modelling the emission of gravitational waves
The prediction of gravitational waves dates back to the year 1916. The first indirect evidence was found in 1974 when observations with radio telescopes demonstrated that a binary system comprising two pulsars (i.e. rotating neutron stars) looses energy at a rate compatible with the emission of gravitational waves. In 2015, the earth-based LIGO gravitational wave detector recorded a signal resulting from the merger of two black holes. The discoveries in 1974 and 2015 were awarded with the Nobel Prize in physics. Signals from mergers of two neutron stars (as shown on the poster) or from mergers of a neutron star and a black hole have also been detected. There a several projects for gravitational wave detector on Earth (e.g. the Einstein telescope) and in space (e.g. LISA).
Poster 6
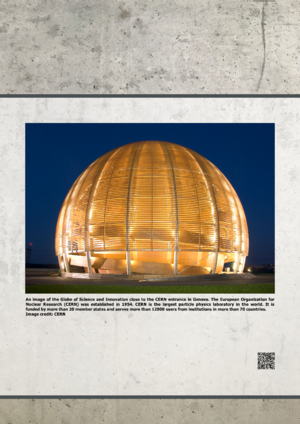
The Globe of Science and Innovation near the CERN entrance in Geneva
The European Organization for Nuclear Research (CERN) was founded 1954 to enhance science and unite European scientists. Its convention explicitly states its non-militaristic purpose. Since its foundation, CERN has grown and serves as a science hub for over 15000 users and employees worldwide. Among the greatest achievements are the discovery of the neutral currents, W, Z, and Higgs bosons, and the first creation of anti-hydrogen (that is hydrogen atoms but made out of antimatter). Also, the world-wide used web protocol “www” originates from CERN. CERN is open to visitors from all over the world. The pictured Globe of Science and Innovation is a museum just at the entrance of the laboratory. In 2023, CERN added the CERN gateway with additional exhibitions, shops, and a restaurant cafe.
Poster 7
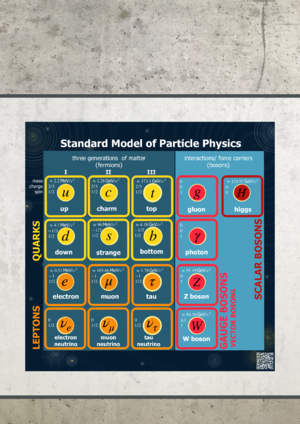
Particles of the Standard Model of particle physics
The particle content of the Standard Model comprises 12 fundamental fermions with spin 1/2, namely six quarks, three charged leptons and three neutrinos. The fundamental fermions are arranged in three families, and only particles from the first family make up all the atoms around us. The forces between particles (electro-weak, strong) are mediated by gauge bosons (gluon, photon, Z boson, W bosons) with spin 1. The Higgs boson has spin 0 and a mass of about 125 GeV. All other particles attain their masses by the interaction with the omnipresent Higgs field. More online information about the fundamental particles and the numerous particles which are bound states of fundamental fermions can be found at the web pages of the Particle Data Group.
Poster 8
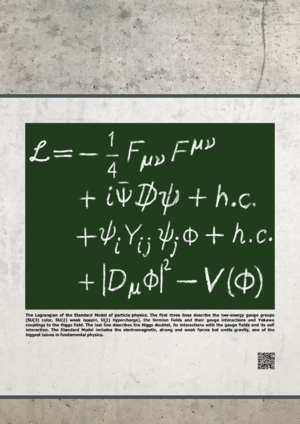
The Lagrangian of the Standard Model of particle physics
The Standard Model (SM) is a quantum field theory summarising our current knowledge of the fundamental particles and their interactions - except gravity. Many of its predictions (like the existence of the Higgs boson) were confirmed by experiments, some of them with astounding precision (e.g. the value of the anomalous magnetic dipole moment of the electron). There are, however, good reasons to assume that the model is incomplete. The SM has a number of free parameters and can not account for phenomena like dark matter or dark energy. Searches for Physics Beyond the Standard Model are therefore an important field of research. Such searches are conducted at particle colliders (e.g. in the context of the ATLAS experiment at the LHC) and in high-precision laboratory experiments (e.g. with the help of highly-charged ion clocks).
Poster 9
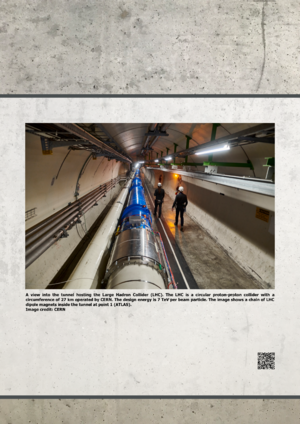
A view into the tunnel hosting the Large Hadron Collider (LHC)
The Large Hadron Collider (LHC) is the world’s biggest and most powerful particle accelerators located in a 27 km long circular tunnel, pictured in this poster, over a hundred meters below the surface. It is the last stage of a whole complex of accelerators, accelerating protons to energies of more than 7000 times their own rest energy. The acceleration at the LHC happens at eight radiofrequency cavities per beam. They have a frequency of 400 MHz and protons pass through them more than 10 million times to reach their final energy. The protons are kept on a circular path by thousands of 15m long dipole superconducting magnets, each with an magnetic field of about 8 Tesla and cooled to about -271○C. Other important magnets at the LHC are the 392 quadrupole magnets that focus the proton beams.
Poster 10
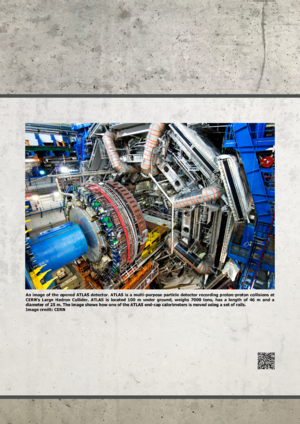
An image of the opened ATLAS detector
The image shows one of the ATLAS end-cap calorimeters being inserted into the experiment in 2009. The ATLAS experiment is one of the four big experiments at CERN’s Large Hadron Collider. It is located 100m underground, weighs 7000 tons, has a length of 46 m and a diameter of 25 m. The experiment consists of several components: three inner tracking detectors, two calorimeters, and a muon tracker, as well as several toroidal magnets and a 2T solenoid. ATLAS is one of the two experiments that discovered the Higgs boson in 2012. Being a general-purpose particle detector, ATLAS can study all known particles including the top quarks, the heaviest fundamental particle we know, but also physics beyond our current knowledge are studied. At Humboldt-University, we study the Higgs self-coupling and search for heavy neutral leptons.
Poster 11
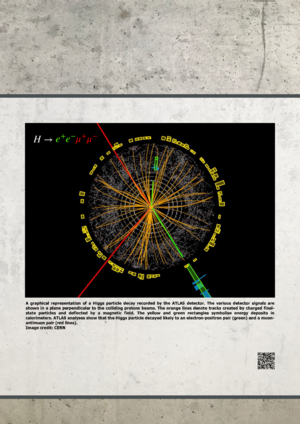
A graphical representation of Higgs particle decay
The picture shows a graphical representation of a Higgs boson candidate, as it decays into a muon-antimuon (red objects) and an electron-positron pair (green objects). Both pairs are measured by the ATLAS experiment. Through conservation of momentum and energy, physicists can deduct the properties of the Higgs boson such as its mass by measuring the kinematic properties of its decay products (in this case of measuring all four objects). Together with further measurements of these objects, scientists have proven the existence of the Higgs boson and thus prove the mechanism of how fundamental particles obtain their mass. One outstanding measurement is the coupling of the Higgs field with itself. Scientists at Humboldt-University are actively involved in achieving that measurement.
Poster 12
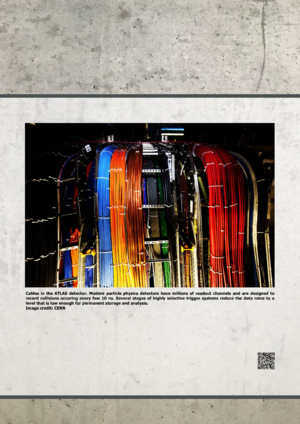
Cables in the ATLAS detector
The large hadron collider (LHC) creates collisions every 25 ns. In these collisions, up to several thousands particles are produced. We would need to store 60 TB of data per second, and need many millions CPU cores to process all the data. This is unfeasible. But as the vast majority of collisions are low-energy physics that has been studied with previous experiments, only a tiny fraction of collisions is worth to be studied. In the ATLAS experiment, events are selection with a two-tiered trigger system: The first stage is based with dedicated hardware built into the detector. The selected data are then transported off the detector for further analysis through cables and fibers like the ones shown in this poster. In the second stage, a computer farm with dedicated software creates a more precise view of the events using algorithms very similar to those of the final reconstruction software. This reduces the amount of data processed and stored by a factor of 40000.
Poster 13
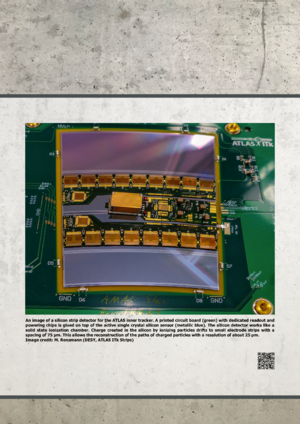
An image of a silicon strip detector for the ATLAS inner tracker
The Inner Tracker of the ATLAS experiment will consist of thousands of detector modules like the one shown on the poster. The detector modules will be arranged in several layers, allowing the very precise three-dimensional reconstruction of the flight paths of charged particles, which are created in the collision of ultrarelativistic protons in the LHC. Inside the silicon sensors, the electrons and holes created by the energetic charged particles drift in an electric field and induce a current signal in thin electrode strips with a spacing of 75 μm. By reading out the signal of each individual electrode strip, the paths of charged particles can be reconstructed with a resolution of 25 μm for a single module and even better for the final, layered detector.
Poster 14
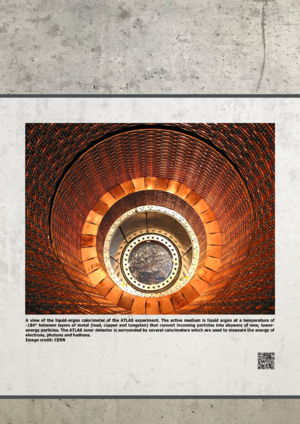
A view of the liquid-argon calorimeter of the ATLAS experiment
Calorimeters are detectors to measure the energy of particles: highly energetic particles are absorbed in a heavy material which then re-emits several lower-energy particles. Multiple of these conversions lead to a shower of many low-energetic particles. These are then measured in an active material. In the ATLAS experiment, one such calorimeter is the pictured the liquid argon calorimeter: layers of metal (copper, lead, and tungsten) are interleaved with regions of liquid argon that is cooled down to -184○C. The low-energy particles of the shower ionize the argon, creating an electric current. By measuring this current, one can identify the energy of the original particle. In order to avoid cracks and holes in the calorimeter, a special design of the liquid argon calorimeter is used: its structure is accordion-like with a honeycomb pattern.
Poster 15
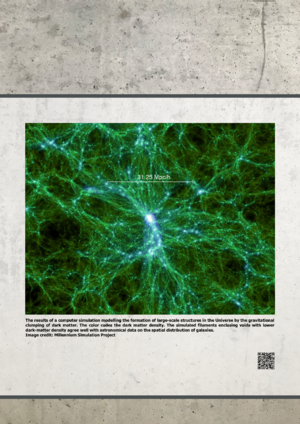
Result of a structure-formation simulation
The Millenium Simulation Project conducted a series of computer simulations tracing how the gravitational clumping of matter results in the large-scale structure (i.e. the distribution of galaxies and voids) observed in the universe. The simulations included only dark matter and no baryons; they employed typically 1010 pseudo-particles with masses of 109 solar masses to investigate the formation of some 107 galaxies in a selected volume.
Poster 16
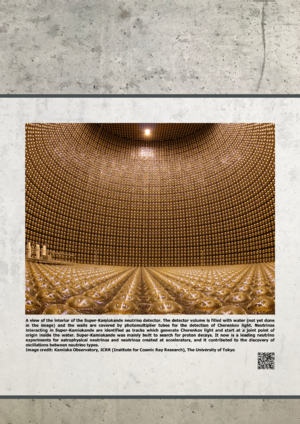
A view of the interior of the Super-Kamiokande neutrino detector
The Super-Kamiokande is an underground neutrino observatory in Japan. It consists of 50'000 tons of water in a cavern whose walls are covered with photomultipliers for the detection of Cherenkov light. The location in a depth of about 1 km and several veto layers faciliate the reduction of backgrounds. Neutrinos interacting in the water generate charged particles whose Cherenkov emission is detected by the photomultipliers. The detected light distribution is indicative of the type of neutrino. Super-Kamiokande had predecessors (in particular Kamiokande II) that detected neutrinos released by the supernova 1987a and demonstrated that the sun is a neutrino source. Super-Kamiokande announced the discovery of neutrino oscillations in 1998. Plans for an even bigger observatory (Hyper-Kamiokande) have been approved.
Poster 17
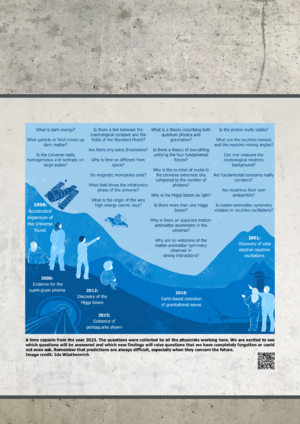
A time capsule from the year 2023
This poster is the result of a survey among the particle physicists working at HU in the year 2023. We collected questions that we would like to see answered in the next years. It is quite likely that it will be embarrassing to read this poster in some years from now :-)
Contributors: Cigdem Issever, Heiko Lacker, Thomas Lohse, Christian Scharf, Ullrich Schwanke, Hannsjörg Weber
Poster Design: Ida Wöstheinrich
This is the link to poster 1
This is the link to poster 2
This is the link to poster 3
This is the link to poster 4
This is the link to poster 5
This is the link to poster 6
This is the link to poster 7
This is the link to poster 8
This is the link to poster 9
This is the link to poster 10
This is the link to poster 11
This is the link to poster 12
This is the link to poster 13
This is the link to poster 14
This is the link to poster 15
This is the link to poster 16
This is the link to poster 17